The Vital Question: Energy, Evolution, and the Origins of Complex Life
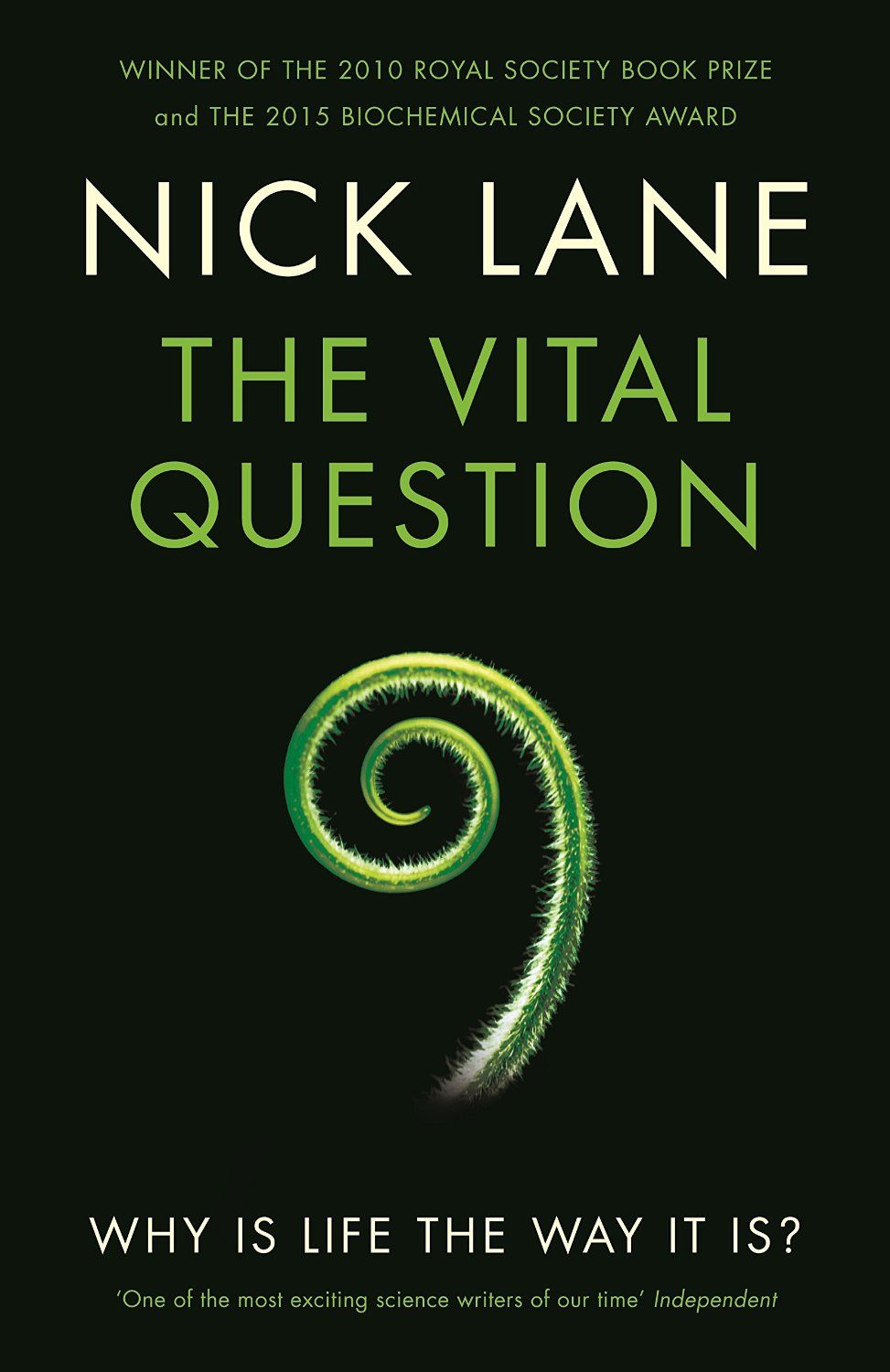
"The Vital Question" purports to fill the "black hole at the heart of biology" - how did complex life evolve? By taking an energy-focused perspective, Lane constructs an argument that mitochondria can explain the origin of complex life and lots of other aspects of life too - sex, death, and aging. I found his arguments generally persuasive - if you're going to hang your hat on something, the second law of thermodynamics isn't a bad option.
If everything he says in here is true, this seems like a big leap forward. I'm not a subject-matter expert myself though, so it will be interesting to see the response from the scientific community. At least he has testable hypotheses!
I'd hesitate to recommend this book to a general audience. I had a rough time making it through this one and I was straining to remember things from my high school AP biology class. Lane does try to spice things up a bit, but he was too melodramatic and overfond of biblical quotations. But overall, he does a pretty good job of making a wide range of scientific areas understandable and I certainly had a few "oh wow" moments.
I'd also recommend checking out Bill Gates' review and video at https://www.gatesnotes.com/Books/The-Vital-Question
My highlights below:
INTRODUCTION: WHY IS LIFE THE WAY IT IS?
There is a black hole at the heart of biology. Bluntly put, we do not know why life is the way it is. All complex life on earth shares a common ancestor, a cell that arose from simple bacterial progenitors on just one occasion in 4 billion years. Was this a freak accident, or did other ‘experiments’ in the evolution of complexity fail? We don’t know.
Life arose around half a billion years after the earth’s formation, perhaps 4 billion years ago, but then got stuck at the bacterial level of complexity for more than 2 billion years, half the age of our planet. Indeed, bacteria have remained simple in their morphology (but not their biochemistry) throughout 4 billion years. In stark contrast, all morphologically complex organisms – all plants, animals, fungi, seaweeds and single-celled ‘protists’ such as amoeba – descend from that singular ancestor about 1.5–2 billion years ago.
There are no surviving evolutionary intermediates, no ‘missing links’ to give any indication of how or why these complex traits arose, just an unexplained void between the morphological simplicity of bacteria and the awesome complexity of everything else. An evolutionary black hole.
And then came the first of three major revolutions that have wracked our view of life in the past half century. This first was instigated by Lynn Margulis in the summer of love, 1967. Complex cells did not evolve by ‘standard’ natural selection, Margulis argued, but in an orgy of cooperation, in which cells engaged with each other so closely that they even got inside each other. NOTE: endosymbiosis
Revolution number two was the phylogenetic revolution – the ancestry of genes.
Ribosomes have an error rate of about one letter in 10,000, far lower than the defect rate in our own high-quality manufacturing processes. And they operate at a rate of about 10 amino acids per second, building whole proteins with chains comprising hundreds of amino acids in less than a minute.
Woese chose one of the subunits from the ribosome, a single machine part, so to speak, and compared its sequence across different species, from bacteria such as E. coli to yeast to humans. His findings were a revelation, and turned our world view on its head. He could distinguish between the bacteria and complex eukaryotes without any difficulty, laying out the branching tree of genetic relatedness within and between each of these magisterial groups. The only surprise in this was how little difference there is between plants and animals and fungi, the groups that most biologists have spent most of their lives studying. What nobody anticipated was the existence of a third domain of life.
Woese’s revolution was real; but his prescription for holism, taking whole organisms and full genomes into account, is right now ushering in the third cellular revolution – and it overturns Woese’s own. This third revolution isn’t over yet. It’s a little more subtle in reasoning, but packs the biggest punch of all. It is rooted in the first two revolutions, and specifically in the question: how do the two relate? Woese’s tree depicts the divergence of one fundamental gene in the three domains of life. Margulis, in contrast, has genes from different species converging together in the mergers and acquisitions of endosymbiosis.
This radical proposition – complex life arose from a singular endosymbiosis between an archaeon host cell and the bacteria that became mitochondria – was predicted by the brilliantly intuitive and free-thinking evolutionary biologist Bill Martin, in 1998, on the basis of the extraordinary mosaic of genes in eukaryotic cells, a mosaic largely uncovered by Martin himself.
The salient point is: Martin predicted that complex life arose through a singular endosymbiosis between two cells only. He predicted that the host cell was an archaeon, lacking the baroque complexity of eukaryotic cells. He predicted that there never was an intermediate, simple eukaryotic cell, which lacked mitochondria; the acquisition of mitochondria and the origin of complex life was one and the same event. And he predicted that all the elaborate traits of complex cells, from the nucleus to sex to phagocytosis, evolved after the acquisition of mitochondria, in the context of that unique endosymbiosis. This is one of the finest insights in evolutionary biology, and deserves to be much better known.
I believe the clue lies in the bizarre mechanism of biological energy generation in cells. This strange mechanism exerts pervasive but little appreciated physical constraints on cells. Essentially all living cells power themselves through the flow of protons (positively charged hydrogen atoms), in what amounts to a kind of electricity – proticity – with protons in place of electrons.
The use of cross-membrane proton gradients to power cells was utterly unanticipated. First proposed in 1961 and developed over the ensuing three decades by one of the most original scientists of the twentieth century, Peter Mitchell, this conception has been called the most counterintuitive idea in biology since Darwin, and the only one that compares with the ideas of Einstein, Heisenberg and Schrödinger in physics. At the level of proteins, we now know how proton power works in detail. We also know that the use of proton gradients is universal across life on earth – proton power is as much an integral part of all life as the universal genetic code. Yet we know next to nothing about how or why this counterintuitive mechanism of energy harnessing first evolved. So it seems to me there are two big unknowns at the very heart of biology today: why life evolved in the perplexing way it did, and why cells are powered in such a peculiar fashion.
It can be frowned upon to act as an advocate in science, but there is a fine tradition of doing exactly that in biology, going back to Darwin himself; he called The Origin of Species ‘one long argument’. A book is still the best way to lay out a vision of how facts might relate to each other across the whole fabric of science – a hypothesis that makes sense of the shape of things. Peter Medawar described a hypothesis as an imaginative leap into the unknown. Once the leap is taken, a hypothesis becomes an attempt to tell a story that is understandable in human terms. To be science, the hypothesis must make predictions that are testable. There’s no greater insult in science than to say that an argument is ‘not even wrong’, that it is invulnerable to disproof.
Andrew Pomiankowski, a mathematically minded evolutionary geneticist and best of colleagues at University College London; and with several extremely able PhD students.
For me the best books in biology, ever since Darwin, have been arguments. This book aspires to follow in that tradition. I will argue that energy has constrained the evolution of life on earth; that the same forces ought to apply elsewhere in the universe; and that a synthesis of energy and evolution could be the basis for a more predictive biology, helping us understand why life is the way it is, not only on earth, but wherever it might exist in the universe.
PART I - THE PROBLEM
1 - WHAT IS LIFE?
Forty years ago, at the dawn of molecular biology, the French biologist Jacques Monod wrote his famous book Chance and Necessity, which argues bleakly that the origin of life on earth was a freak accident, and that we are alone in an empty universe. The final lines of his book are close to poetry, an amalgam of science and metaphysics: The ancient covenant is in pieces; man knows at last that he is alone in the universe’s unfeeling immensity, out of which he emerged only by chance. His destiny is nowhere spelled out, nor is his duty. The kingdom above or the darkness below: it is for him to choose.
Life on earth is not really a sample of one, but for practical purposes it is an infinite variety of organisms evolving over infinite time. Yet evolutionary theory does not predict, from first principles, why life on earth took the course that it did.
My argument in this book is that there are in fact strong constraints on evolution – energetic constraints – which do make it possible to predict some of the most fundamental traits of life from first principles. Before we can address these constraints, we must consider why evolutionary biology is not predictive, and why these energetic constraints have passed largely unnoticed; indeed, why we have hardly even noticed there is a problem.
To a point, we can blame DNA for this sorry state of affairs. Ironically, the modern era of molecular biology, and all the extraordinary DNA technology that it entails, arguably began with a physicist, specifically with the publication of Erwin Schrödinger’s book What is Life? in 1944. Schrödinger made two key points: first, that life somehow resists the universal tendency to decay, the increase in entropy (disorder) that is stipulated by the second law of thermodynamics; and second, that the trick to life’s local evasion of entropy lies in the genes.
A tiny proportion of our own genome, less than 2%, codes for proteins; a larger portion is regulatory; and the function of the rest is liable to cause intemperate rows among otherwise polite scientists.
Onions, wheat and amoebae have more genes and more DNA than we do. Amphibians such as frogs and salamanders have genome sizes that range over two orders of magnitude, with some salamander genomes being 40 times larger than our own, and some frogs being less than a third of our size.
Reading the make-up of a long-vanished world from a few scattered zircon crystals puts a lot of weight on what amounts to grains of sand, but it is better than no evidence at all. That evidence consistently conjures up a planet that was surprisingly similar to the one we know today. The occasional asteroid impact might have partially vaporised the oceans, but is unlikely to have upset any bacteria living in the deep oceans – if they had already evolved.
Beyond these direct fossils, by 3.2 billion years ago there are large-scale geological features, hundreds of square miles in area and tens of metres deep, notably banded iron formations and carbon-rich shales. We tend to think of bacteria and minerals as occupying different realms, living versus inanimate, but in fact many sedimentary rocks are deposited, on a colossal scale, by bacterial processes.
The old version sees oxygen as the critical environmental determinant of life. Oxygen does not specify what will evolve, the argument goes, but it permits the evolution of far greater complexity – it releases the brakes. Animals, for example, make their living by physically moving around, chasing prey or being chased themselves. Obviously this requires a lot of energy, so it’s easy to imagine that animals could not exist in the absence of oxygen, which provides nearly an order of magnitude more energy than other forms of respiration.
Biology is all about genes, and their behaviour is all about the environment. What else is there, after all? Well, biology is not only about genes and environment, but also cells and the constraints of their physical structure, which we shall see have little to do with either genes or environment directly. The predictions that arise from these disparate world views are strikingly different.
To put that more formally, if rising oxygen levels permitted flourishing new life styles, we would expect to see a polyphyletic radiation, in which unrelated cells or organisms (from different phyla) adapt swiftly, radiating new species that fill unoccupied niches. This kind of pattern is exactly what we do see – sometimes.
If complex eukaryotic cells really did evolve in response to the rise in atmospheric oxygen, we would predict a polyphyletic radiation, with various different groups of bacteria begetting more complex cell types independently.
It’s a poetic notion, but the serial endosymbiosis theory makes an implicit prediction equivalent to that of standard selection. If it were true, we would expect to see polyphyletic origins – a mixed bag of internal structures, as varied as the external appearance of cells.
The fact that all eukaryotes have mitochondria may seem to be a trivial point, but when combined with the proliferation of genome sequences from across the wider microbial world, this knowledge has turned our understanding of eukaryotic evolution on its head.
We now know that eukaryotes all share a common ancestor, which by definition arose just once in the 4 billion years of life on earth. Let me reiterate this point, as it is crucial. All plants, animals, algae, fungi and protists share a common ancestor – the eukaryotes are monophyletic. This means that plants did not evolve from one type of bacteria, and animals or fungi from other types. On the contrary, a population of morphologically complex eukaryotic cells arose on a single occasion – and all plants, animals, algae and fungi evolved from this founder population. Any common ancestor is by definition a singular entity – not a single cell, but a single population of essentially identical cells.
The killer fact that emerges from this enormous diversity is how damned similar eukaryotic cells are. We do not find all kinds of intermediates and unrelated variants. The prediction of the serial endosymbiosis theory, that we should, is wrong.
2 - WHAT IS LIVING?
So the release of heat when oil and water separate actually increases entropy. In terms of overall entropy, then, and taking all these physical interactions into consideration, an ordered oily membrane around a cell is a higher entropy state than a random mixture of immiscible molecules, even though it looks more ordered.
Perhaps most surprisingly, there is little difference in overall entropy between a disordered soup of individual amino acids (the building blocks of proteins) and a beautifully folded protein.
The idea that life is a low-entropy state – that it is more organised than a soup – is not strictly true. The order and organisation of life is more than matched by the increased disorder of the surroundings.
Because organisms are ‘out of equilibrium’ with the oxygenated global environment, they will tend to oxidise, unless the process is actively prevented.
Thus, ultimately, the power for growth comes from the reactivity of the environment, which fluxes continuously through living cells (in the form of food and oxygen in our case, photons of light in the case of plants). Living cells couple this continuous energy flux to growth, overcoming their tendency to break down again. They do so through ingenious structures, in part specified by genes. But whatever those structures may be (we’ll come to that), they are themselves the outcome of growth and replication, natural selection and evolution, none of which is possible in the absence of a continuous energy flux from somewhere in the environment.
A single cell consumes around 10 million molecules of ATP every second! The number is breathtaking. There are about 40 trillion cells in the human body, giving a total turnover of ATP of around 60–100 kilograms per day – roughly our own body weight. In fact, we contain only about 60 grams of ATP, so we know that every molecule of ATP is recharged once or twice a minute.
Bacteria such as E. coli can divide every 20 minutes. To fuel its growth E. coli consumes around 50 billion ATPs per cell division, some 50–100 times each cell’s mass. That’s about four times our own rate of ATP synthesis. Convert these numbers into power measured in watts and they are just as incredible. We use about 2 milliwatts of energy per gram – or some 130 watts for an average person weighing 65 kg, a bit more than a standard 100 watt light bulb. That may not sound like a lot, but per gram it is a factor of 10,000 more than the sun (only a tiny fraction of which, at any one moment, is undergoing nuclear fusion). Life is not much like a candle; more of a rocket launcher.
In the end, respiration and burning are equivalent; the slight delay in the middle is what we know as life.
Life could have been driven by thermal or mechanical energy, or radioactivity, or electrical discharges, or UV radiation, the imagination is the limit; but no, all life is driven by redox chemistry, via remarkably similar respiratory chains.
For each pair of electrons stripped from food, ten protons are ferried across the membrane. And that’s it (Figure 9). A little less than half the energy released by flow of electrons to oxygen is saved in the proton gradient. All that power, all that ingenuity, all the vast protein structures, all of that is dedicated to pumping protons across the inner mitochondrial membrane.
The ATP synthase and the proton-motive force are also conserved universally across life. And I mean universally. The ATP synthase is found in basically all bacteria, all archaea, and all eukaryotes (the three domains of life we discussed in the previous chapter), barring a handful of bugs that rely on fermentation instead. It is as universal as the genetic code itself.
With private means and a practical bent, Mitchell spent two years refurbishing a manor house near Bodmin in Cornwall as a lab and home, and opened the Glynn Institute there in 1965. For the next two decades, he and a small number of other leading figures in bioenergetics set about testing the chemiosmotic hypothesis to destruction.
This is the stuff of legend: a nice example of how science works in unexpected ways, touted as a ‘paradigm shift’ in biology supporting Thomas Kuhn’s view of scientific revolutions, but now safely confined to the history books. The details have been worked out at atomic resolution, culminating in John Walker’s Nobel Prize in 1997 for the structure of the ATP synthase.
Our modern focus on molecular biology means we have all but forgotten Mitchell’s preoccupation with membranes as a necessary link between inside and outside, with what Mitchell called ‘vectorial chemistry’ – chemistry with a direction in space, where position and structure matter. Not test-tube chemistry, where everything is mixed in solution.
Consider the problem the other way around: what is good about the redox chemistry of respiration? Plenty, it seems. When I say respiration, we need to look beyond ourselves. We strip electrons from food and transfer them down our respiratory chains to oxygen, but the critical point here is that the source and the sink of electrons can both be changed. It so happens that burning up food in oxygen is as good as it gets in terms of energy yield, but the underlying principle is enormously wider and more versatile. There is no need to eat organic matter, for example. Hydrogen gas, hydrogen sulphide and ferrous iron are all electron donors, as we’ve already noted. They can pass their electrons into a respiratory chain, so long as the acceptor at the other end is a powerful enough oxidant to pull them through. That means bacteria can ‘eat’ rocks or minerals or gases, using basically the same protein equipment that we use in respiration.
There is about the same metabolic versatility in the entire eukaryotic domain – all plants, animals, algae, fungi and protists – as there is in a single bacterial cell.
The fact that many electron donors and acceptors are both soluble and stable, entering and exiting cells without much ado, means that the reactive environment required by thermodynamics can be brought safely inside, right into those critical membranes. That makes redox chemistry much easier to deal with than heat or mechanical energy, or UV radiation or lightning, as a form of biologically useful energy flux.
The great advantage of respiration, then, is its immense versatility. Essentially any redox couple (any pair of electron donor and electron acceptor) can be used to set electrons flowing down respiratory chains.
Take DNA replication, which we might guess would be as fundamental to life as the genetic code. Yet the detailed mechanisms of DNA replication, including almost all the enzymes needed, turn out to be totally different in bacteria and archaea. Likewise, the cell wall, the rigid outer layer that protects the flimsy cell inside, is chemically completely different in bacteria and archaea. So are the biochemical pathways of fermentation. Even the cell membranes – strictly necessary for chemiosmotic coupling, otherwise known as membrane bioenergetics – are biochemically different in bacteria and archaea. In other words, the barriers between the inside and outside of cells and the replication of hereditary material are not deeply conserved. What could be more important to the lives of cells than these! In the face of all that divergence, chemiosmotic coupling is universal.
Evolution should tend to play out along similar lines, guided by similar constraints, elsewhere in the universe. If I am right (and I don’t for a moment think I will be in all the details, but I hope that the bigger picture is correct) then these are the beginnings of a more predictive biology. One day it may be possible to predict the properties of life anywhere in the universe from the chemical composition of the cosmos.
A more human example is the Vasa, a formidable seventeenth-century Swedish warship that sank in the bay outside Stockholm on its maiden voyage in 1628, and was salvaged in 1961. It had been wonderfully preserved as the growing city of Stockholm poured its sewage into the marine basin. It was literally preserved in shit, with the sewer gas hydrogen sulphide preventing oxygen from attacking the ship’s exquisite wooden carving. Ever since raising the ship, it has been a fight to keep it intact.
PART II THE ORIGIN OF LIFE
3 - ENERGY AT LIFE’S ORIGIN
From an energetic point of view, the power of enzymes is not so much that they speed up reactions, but that they channel their force, maximising the output.
What is missing here is energy. Of course, energy figures in the primordial soup – all those flashes of lightning. I once calculated that to sustain a tiny primitive biosphere, equivalent in size to that before the evolution of photosynthesis, by lightning alone, would require four bolts of lightning per second, for every square kilometre of ocean. And that’s assuming a modern efficiency of growth.
All living organisms are sustained by far-from-equilibrium conditions in their environment: we, too, are dissipative structures. The continuous reaction of respiration provides the free energy that cells need to fix carbon, to grow, to form reactive intermediates, to join these building blocks together into long-chain polymers such as carbohydrates, RNA, DNA and proteins, and to maintain their low-entropy state by increasing the entropy of the surroundings. In the absence of genes or information, certain cell structures, such as membranes and polypeptides, should form spontaneously, so long as there is a continuous supply of reactive precursors – activated amino acids, nucleotides, fatty acids; so long as there is a continuous flux of energy providing the requisite building blocks. Cell structures are forced into existence by the flux of energy and matter. The parts can be replaced but the structure is stable and will persist for as long as the flux persists. This continuous flux of energy and matter is precisely what is missing from the primordial soup. There is nothing in soup that can drive the formation of the dissipative structures that we call cells, nothing to make these cells grow and divide, and come alive, all in the absence of enzymes that channel and drive metabolism.
What does it take to make a cell? Six basic properties are shared by all living cells on earth. Without wishing to sound like a textbook, let’s just enumerate them. All need: (i) a continuous supply of reactive carbon for synthesising new organics; (ii) a supply of free energy to drive metabolic biochemistry – the formation of new proteins, DNA, and so on; (iii)catalysts to speed up and channel these metabolic reactions; (iv)excretion of waste, to pay the debt to the second law of thermodynamics and drive chemical reactions in the correct direction; (v) compartmentalisation – a cell-like structure that separates the inside from the outside; (vi) hereditary material – RNA, DNA or an equivalent, to specify the detailed form and function.
Nietzsche once observed that humans will not mistake ourselves for gods so long as we need to defecate. But in fact excretion is a thermodynamic necessity, binding even for the godliest. For any reaction to continue in a forward direction, the end product must be removed.
Black smokers are also very unstable, growing and collapsing over a few decades at most. That’s not long to ‘invent’ life. While they are truly far-from-equilibrium dissipative structures, and certainly solve some of the problems of soup, these volcanic systems are too extreme and unstable to nurture the gentle carbon chemistry needed for the origin of life. What they did do, which was indispensable, was charge the early oceans with catalytic metals such as ferrous iron (Fe2+) and nickel (Ni2+) derived from magma.
Alkaline hydrothermal vents provide exactly the conditions required for the origin of life: a high flux of carbon and energy that is physically channelled over inorganic catalysts, and constrained in a way that permits the accumulation of high concentrations of organics.
The combination of high CO2, mildly acidic oceans, alkaline fluids, and thin, FeS-bearing vent walls is crucial, because it promotes chemistry that would otherwise not happen easily.
But now think of a proton gradient across a membrane. The proton concentration – the acidity – is different on opposite sides of the membrane. Exactly the same difference is found in alkaline vents. Alkaline hydrothermal fluids wend their way through the labyrinth of micropores. So do mildly acidic ocean waters. In some places there is a juxtaposition of fluids, with acidic ocean waters saturated in CO2 separated from alkaline fluids rich in H2 by a thin inorganic wall, containing semiconducting FeS minerals. The reduction potential of H2 is lower in alkaline conditions: it desperately ‘wants’ to be rid of its electrons, so the left-over H+ can pair up with the OH– in the alkaline fluids to form water, oh so stable. At pH 10, the reduction potential of H2 is –584 mV: strongly reducing. Conversely, at pH 6, the reduction potential for formate is –370m V, and for formaldehyde it is –520m V. In other words, given this difference in pH, it is quite easy for H2 to reduce CO2 to make formaldehyde. The only question is: how are electrons physically transferred from H2 to CO2? The answer is in the structure. FeS minerals in the thin inorganic dividing walls of microporous vents conduct electrons. They don’t do it nearly as well as a copper wire, but they do it, nonetheless. And so in theory, the physical structure of alkaline vents should drive the reduction of CO2 by H2, to form organics (Figure 14). Fantastic!
But alkaline vents come with both a serious problem and a beautiful answer to the problem. The serious problem is that these vents are rich in hydrogen gas, but hydrogen will not react with CO2 to form organics. The beautiful answer is that the physical structure of alkaline vents – natural proton gradients across thin semiconducting walls – will (theoretically) drive the formation of organics. And then concentrate them. To my mind, at least, all this makes a great deal of sense. Add to this the fact that all life on earth uses (still uses!) proton gradients across membranes to drive both carbon and energy metabolism, and I’m tempted to cry, with the physicist John Archibald Wheeler, ‘Oh, how could it have been otherwise! How could we all have been so blind for so long!’ Let’s calm down and finish.
4 - THE EMERGENCE OF CELLS
The acetyl CoA pathway starts with hydrogen and carbon dioxide – the same two molecules that we discussed in the last chapter as being plentiful in alkaline hydrothermal vents. As we noted then, the reaction between CO2 and H2 to form organics is exergonic, which is to say that it releases energy: in principle the reaction should take place spontaneously. In practice, there is an energetic barrier that prevents H2 and CO2 from reacting quickly. Methanogens use the proton gradient to overcome this barrier, which I shall argue was the ancestral state. Be that as it may, methanogens and acetogens both power their growth through the reaction of H2 and CO2 alone: that reaction provides all the carbon and all the energy needed for growth. This sets the acetyl CoA pathway apart from the other five pathways of carbon fixation. The geochemist Everett Shock summed it up memorably as ‘a free lunch that you’re paid to eat’. It may be a meagre lunch, but in the vents it’s served all day.
It is almost as hard to define death as life, but the irrevocable collapse of membrane potential comes pretty close.
In short, unless there is a pump that can get rid of protons from inside the cell, natural proton gradients can’t drive anything. They equilibrate, and equilibrium is death.
Taking concentration differences and charge into consideration, as well as the operation of proteins like the ATP synthase, we showed that only cells with very leaky membranes can use natural proton gradients to power carbon and energy metabolism. Remarkably, these leaky cells theoretically glean as much energy from a natural proton gradient of 3 pH units as modern cells gain from respiration.
There are several surprising ramifications of this simple invention. One is almost incidental: pumping sodium out of the cell lowers the concentration of sodium within the cell. We know that many core enzymes found in both bacteria and archaea (those responsible for transcription and translation, for example) have been optimised by selection to work at low Na+ concentration, despite most probably evolving in the oceans, where the Na+ concentration seems to have been high even 4 billion years ago. The early operation of an antiporter could potentially explain why all cells are optimised to low sodium, despite evolving in a high-sodium environment.
PART III COMPLEXITY
5 - THE ORIGIN OF COMPLEX CELLS
Yet throughout these shifts, the bacteria remained resolutely bacterial. Never did they give rise to something as large and complex as a flea. Nothing is more conservative than a bacterium.
That may seem an absurd fantasy, but it is close to the situation that confronts us in biology at the moment. There really are no intermediate ‘civilisations’ between bacteria and eukaryotes.
Here’s what’s interesting. Different genes in the same eukaryotic organism do not all share the same ancestor. Around three-quarters of eukaryotic genes that have prokaryotic homologues apparently have bacterial ancestry, whereas the remaining quarter seem to derive from archaea. That’s true of humans, but we are not alone. Yeasts are remarkably similar; so too are fruit flies, sea urchins and cycads. At the level of our genomes, it seems that all eukaryotes are monstrous chimeras.
Something odd seems to have happened at the very origin of eukaryotes. It looks like the first eukaryotes picked up thousands of genes from prokaryotes, but then ceased to ply any trade in prokaryotic genes. The simplest explanation for this picture is not bacterial-style lateral gene transfer, but eukaryotic-style endosymbiosis.
This is the simplest possible scenario for the origin of eukaryotes: there was a single chimeric event between an archaeal host cell and a bacterial endosymbiont. I do not expect you to believe me at this point. I am arguing simply that this scenario is compatible with everything we know about the phylogenetic history of eukaryotes, as are several other possible scenarios.
Perhaps surprisingly, fermentation is the only known alternative to chemiosmotic coupling. All forms of respiration, all forms of photosynthesis, and indeed all forms of autotrophy, where cells grow from simple inorganic precursors only, are strictly chemiosmotic.
Despite this basic limitation of chemistry, cells still grow from these redox couples perfectly happily. They do so because proton gradients across membranes are by definition gradations. The beauty of chemiosmotic coupling is that it transcends chemistry. It allows cells to save up ‘loose change’. If it takes 10 protons to make 1 ATP, and a particular chemical reaction only releases enough energy to pump 4 protons, then the reaction can simply be repeated 3 times to pump 12 protons, 10 of which are then used to make 1 ATP.
Proton gradients have become the ultimate sensors of cellular health, the arbiters of life and death, a role that will loom large later in this chapter.
What once seemed to be a peculiar mechanism now looks to be only superficially counterintuitive – our analysis suggests that chemiosmotic coupling ought to be literally a universal property of life in the cosmos.
The issue is energy availability per gene. I had been stumbling blindly towards this concept for some years, but it was the cut and thrust of dialogue with Bill Martin that really brought matters to a head. After weeks of talking, trading ideas and perspectives, it suddenly dawned on us that the key to the evolution of eukaryotes lies in the simple idea of ‘energy per gene’.
By our calculations, eukaryotes have up to 200,000 times more energy per gene than prokaryotes. Two hundred thousand times more energy!
Not much of this extra energy is spent on DNA itself; only about 2% of the overall energy budget of a single-celled organism goes on replicating DNA. In contrast, according to Frank Harold, distinguished elder statesman of microbial bioenergetics (and a hero of mine, even though we don’t always agree), cells spend as much as 80% of their total energy budget on protein synthesis. That’s because cells are mostly made of proteins; about half the dry weight of a bacterium is protein. Proteins are also very costly to make – they are strings of amino acids, usually a few hundred of them linked together in a long chain by ‘peptide’ bonds. Each peptide bond requires at least 5 ATPs to seal, five times as much as is needed to polymerise nucleotides into DNA. And then each protein is produced in thousands of copies, which are continuously turned over to repair wear and tear. To a first approximation, then, the energy costs of cells equate closely to the costs of making proteins.
In other words, eukaryotes can support a genome 5,000 times larger than bacteria, or alternatively, they could spend 5,000 times more ATP on expressing each gene, for example by producing many more copies of each protein; or a mixture of the two, which is in fact the case.
But now think about a population of bacterial endosymbionts. The same general principles apply – this is just another population of bacteria, albeit a small population in a restricted space. Bacteria that lose unnecessary genes will replicate slightly faster and tend to dominate, just as before. The key difference is the stability of the environment. Unlike the great outdoors, where the conditions are always changing, the cytoplasm of cells is a very stable environment. It may not be easy to get there, or to survive there, but once established, a steady and invariable supply of nutrients can be relied upon. The endlessly circular dynamic of gene loss and gain in free-living bacteria is replaced with a trajectory towards gene loss and genetic streamlining. Genes that are not needed will never be needed again. They can be lost for good. Genomes shrink.
Gene loss makes a huge difference. Losing genes is beneficial to the endosymbiont, as it speeds up their replication; but losing genes also saves ATP.
Mitochondria are just as good at making ATP as their free-living ancestors, but they reduced the costly bacterial overheads massively. In effect, eukaryotic cells have multibacteria power, but save on the costs of protein synthesis. Or rather, they divert the costs of protein synthesis.
We need to breathe continuously to stay alive and to exert fine control over muscles in the diaphragm, chest and throat. Down at the level of mitochondria, the mitochondrial genes modulate respiration in much the same way, making sure that output is always finely tailored to demand. No other reason is big enough to explain the universal retention of mitochondrial genes.
There is no benefit in terms of energy per gene from becoming larger, except when large size is attained by endosymbiosis. Only then is gene loss possible, and only then can the shrinking of mitochondrial genomes fuel the expansion of the nuclear genome over several orders of magnitude, up to eukaryotic sizes.
Now we see, too, why natural selection, operating on infinite populations of bacteria over infinite periods of time, should not give rise to large complex cells, what we know as eukaryotes, except by way of a rare and stochastic endosymbiosis.
6 - SEX AND THE ORIGINS OF DEATH
It is time to address the greatest paradox in biology: why it is that all life on earth is divided into prokaryotes, which lack morphological complexity, and eukaryotes, which share a massive number of detailed properties, none of which are found in prokaryotes. There is a gulf, a void, a vacuum, between the two, which nature really ought to abhor.
Only rarely is natural selection a force for change. Most commonly, it opposes change, purging variations from the peaks of an adaptive landscape. Only when that landscape undergoes some kind of seismic shift does selection promote change rather than stasis. And then it can operate startlingly swiftly.
Yet this didn’t happen at the origin of eukaryotes. All eukaryotes share the same set of basic traits. It looks a lot like an interbreeding population. Sex. Could any other form of reproduction have achieved the same end point? I don’t think so. Asexual reproduction – cloning – leads to deep divergence, as different mutations accumulate in different populations.
The fact that eukaryotes share the same traits suggests that they arose in an interbreeding sexual population. This in turn implies that their population was small enough to interbreed. Any cells that did not have sex, in this population, did not survive. The Bible was right: ‘Strait is the gate and narrow is the way, which leadeth unto life, and few there be that find it.’
In sum: sex arose very early in eukaryotic evolution, and only the evolution of sex in a small unstable population can explain why all eukaryotes share so many common traits.
Different proteins can be pieced together from the same gene by differential splicing, enabling the recombinatorial virtuosity of the immune system, for example. Different bits of protein are recombined in marvellous ways to form billions of distinct antibodies, which are capable of binding to practically any bacterial or viral protein, thereby setting in motion the killing machines of the immune system.
The nuclear membrane is a barrier separating transcription from translation – inside the nucleus, genes are transcribed into RNA codescripts; outside the nucleus, the RNAs are translated into proteins on the ribosomes. Crucially, the slow process of splicing takes place inside the nucleus, before the ribosomes can get anywhere near the RNA. That is the whole point of the nucleus: to keep ribosomes at bay. This explains why eukaryotes need a nucleus but prokaryotes don’t – prokaryotes don’t have an intron problem.
The ‘effective’ population size is hugely diminished, making asexual populations far more vulnerable to extinction. That’s exactly what does happen to most asexuals – almost all clonal plants and animals fall extinct within a few million years.
Selection, like God, can now see all our vices and virtues, gene by gene. That’s the great advantage of sex.
Work from the mathematical population geneticists Sally Otto and Nick Barton points to an unholy trinity of factors that conspicuously relates to circumstances at the origin of eukaryotes: the benefits of sex are greatest when the mutation rate is high, selection pressure is strong, and there is a lot of variation in a population.
LOCATION: 3458
In his brilliant book Mendel’s Demon, Mark Ridley compared sex with the New Testament view of sin – just as Christ died for the collected sins of humanity, so too sex can bring together the accumulated mutations of a population into a single scapegoat, and then crucify it.
‘No practical biologist interested in sexual reproduction would be led to work out the detailed consequences experienced by organisms having three or more sexes; yet what else should he do if he wishes to understand why the sexes are, in fact, always two?’ So said Sir Ronald Fisher, one of the founding fathers of evolutionary genetics. The problem has yet to be conclusively solved.
One of the deepest distinctions between the two sexes relates to the inheritance of mitochondria – one sex passes on its mitochondria, while the other sex does not.
This is a fine example of why mathematical population genetics is important: hypotheses need to be tested formally, by whatever methods are possible; in this case, a formal model showed clearly that uniparental inheritance cannot fix in a population unless two mating types already exist. This is as close to rigorous proof as we can get.
In other words, from the simplest of starting points, both anisogamy (distinct gametes, sperm and egg) followed by uniparental inheritance will tend to evolve in organisms with more than one tissue.
What does all this mean? It means, astonishingly, that mitochondrial variation alone can explain the evolution of multicellular organisms that have anisogamy (sperm and eggs), uniparental inheritance, and a germline, in which female germ cells are sequestered early in development – which together form the basis for all sexual differences between males and females. In other words, the inheritance of mitochondria can account for most of the real physical differences between the two sexes.
PART IV - PREDICTIONS
7 - THE POWER AND THE GLORY
Most of the core proteins in the mitochondrial inner membrane, shown in darker shade, are encoded by genes located in the mitochondria themselves. The remaining proteins (lighter shading) are encoded by genes in the nucleus. We have known about this strange state of affairs since the early 1970s, when it first became clear that the mitochondrial genome is so small that it cannot possibly encode most of the proteins found in the mitochondria. The old idea that mitochondria are still independent of their host cells is therefore nonsense. Their ostensible autonomy – they give an eerie impression of replicating themselves whenever they feel like it – is a mirage. The fact is that their function depends on two distinct genomes. They can only grow or function if they are wholly provisioned with proteins encoded by both of these genomes.
Let me ram home just how odd this is. Cell respiration – without which we would die within minutes – depends on mosaic respiratory chains that are composed of proteins encoded by two very different genomes.
Apoptosis makes perfect sense in the context of a multicellular organism. It is necessary for sculpting tissues during embryonic development, and removing and replacing damaged cells. What came as a complete surprise was the central involvement of mitochondria, especially the bona fide respiratory protein cytochrome c. Why on earth would the loss of cytochrome c from the mitochondria act as a signal for cell death?
Once again, as the Russian-born geneticist Theodosius Dobzhansky famously observed, nothing in biology makes sense except in the light of evolution.
The best example comes from Ron Burton, at the Scripps Marine Research Institute, who has been working on mitochondrial–nuclear incompatibilities in the marine copepod Tigriopus californicus for more than a decade.
The sexual barriers between closely related species are far more permeable than the textbooks would have us believe – species that prefer to ignore each other in the wild, for behavioural reasons, will often mate successfully in captivity. The traditional definition of a species – the failure to produce fertile offspring in crosses between populations – is simply not true for many closely related species.
One possible underlying basis is metabolic rate. Even the ancient Greeks appreciated that males are, literally, hotter than females – the ‘hot male’ hypothesis.
All this implies that growth rate is the real force behind sexual development, at least in mammals.
While it’s not always the case that males develop at the higher temperature (for various subtle reasons) the link between sex and growth rate, set either by genes or temperature, is more deeply conserved than any particular mechanism. It does look as if various opportunistic genes have from time to time grabbed the reins of developmental control, setting a developmental rate that induces male or female development. This is one reason, incidentally, why men need not fear the demise of the Y chromosome – its function will probably be taken over by some other factor, possibly a gene on a different chromosome, which sets the faster metabolic rate needed for male development. It might also account for the strange vulnerability of external testicles in mammals; getting the temperature right is much more deeply embedded in our biology than scrota.
Sight is especially vulnerable: the metabolic rate of cells in the retina and optic nerve is the highest in the body, and mitochondrial diseases such as Leber’s hereditary optic neuropathy affect the optic nerve, causing blindness.
Of course, the removal of cells that don’t work well enough only improves overall tissue function if they are replaced with new cells from the stem-cell population. A major problem with neurons and muscle cells is that they cannot be replaced. How could a neuron be replaced? Our life’s experience is written into synaptic networks, each neuron forming as many as 10,000 different synapses. If the neuron dies by apoptosis, those synaptic connections are lost forever, along with all the experience and personality that might have been written into them. That neuron is irreplaceable. In fact, while less obviously necessary, all terminally differentiated tissues are irreplaceable – their very existence is impossible without the deep distinction between germline and soma discussed in the previous chapter. Selection is all about offspring. If organisms with large and irreplaceable brains leave more viable offspring than organisms with small replaceable brains, then they will thrive. Only when there is a distinction between the germline and soma can selection act in this way; but when it does, the body becomes disposable. Lifespan becomes finite. And cells that can’t meet their metabolic requirements will kill us in the end.
The cost of high aerobic capacity is low fertility.
The very fact that most changes from the optimal sequence for flight are eliminated by selection means that less varied mitochondrial DNA remains – so there is less scope for selection to choose some mitochondrial variant that just happens to be particularly good in the cold, or with a fatty diet. It’s curious in this regard that birds frequently migrate rather than suffer a seasonal change in environmental conditions. Could it be that their mitochondria are better able to support the exertion of migration than function in the harsher environment they would face if they stayed put? Conversely, rats have a great deal more variation, and from first principles that should give them the raw material for better adaptation. Does it really?
Fertility. Adaptability. Aerobic fitness. Disease. We can’t cut much closer to the grain of natural selection than that. I reiterate: all these trade-offs emerge inexorably from the requirement for two genomes.
There is one final, indirect cost to having a high death threshold – a faster rate of ageing and a greater propensity to suffer age-related diseases.
A favourite quote of mine is from Barry Halliwell and John Gutteridge, authors of the classic textbook Free Radicals in Biology and Medicine: ‘By the 1990s it was clear that antioxidants are not a panacea for ageing and disease, and only fringe medicine still peddles this notion.’
So why, you may well wonder, do I still think that a more subtle version of the free-radical theory is true? For several reasons. Two critical factors were missing from the original theory: signalling and apoptosis.
In the end, we are optimised by evolution to our expected lifespan. I doubt we will ever find a way of living much beyond 120 by merely fine-tuning our physiology.
From a simple consideration of the requirements for two genomes, we can predict that our ancestors increased their aerobic capacity, decreased their free-radical leak, gave themselves a problem with fertility, and increased their lifespan. How much truth is there in all that? This is a testable hypothesis that might prove wrong.
EPILOGUE: FROM THE DEEP
Incorporating energy into evolution is long overdue, and begins to lay a more predictive basis to natural selection.
How lucky that our minds, the most improbable biological machines in the universe, are now a conduit for this restless flow of energy, that we can think about why life is the way it is. May the proton-motive force be with you!
ACKNOWLEDGEMENTS
I’m also extremely grateful to Professor Steve Jones for his support, and for welcoming me to the department he headed at the time: Genetics, Evolution and Environment, natural home for the research I was to undertake.
The second group of students and post-docs, working on mathematical modelling, have selected themselves from an unparalleled doctoral training programme at UCL, funded until recently by the Engineering and Physical Sciences Research Council. This programme goes by the smart acronym CoMPLEX, standing improbably for the Centre for Mathematics and Physics in the Life Sciences and Experimental Biology. CoMPLEX students working with POM and me include Dr Zena Hadjivasiliou, Victor Sojo, Arunas Radzvilavicius, Jez Owen, and recently, Drs Bram Kuijper and Laurel Fogarty. All started out with rather vague ideas, and turned them into rigorous mathematical models that give striking insights into how biology really works.